Subscribe for the latest discoveries and in-depth analyses in your inbox.
Thank you to our subscribers for your continued support and passion for science!
CRISPR (Clustered Regularly Interspaced Short Palindromic Repeats) has transformed the fields of genetics and molecular biology, enabling precise editing of DNA. Originating from a natural bacterial immune system, CRISPR's journey from its discovery to its application in gene editing represents a fascinating intersection of evolutionary biology and biotechnology. This article explores the evolution of CRISPR, its mechanisms, applications in evolutionary genomics, and its future prospects.
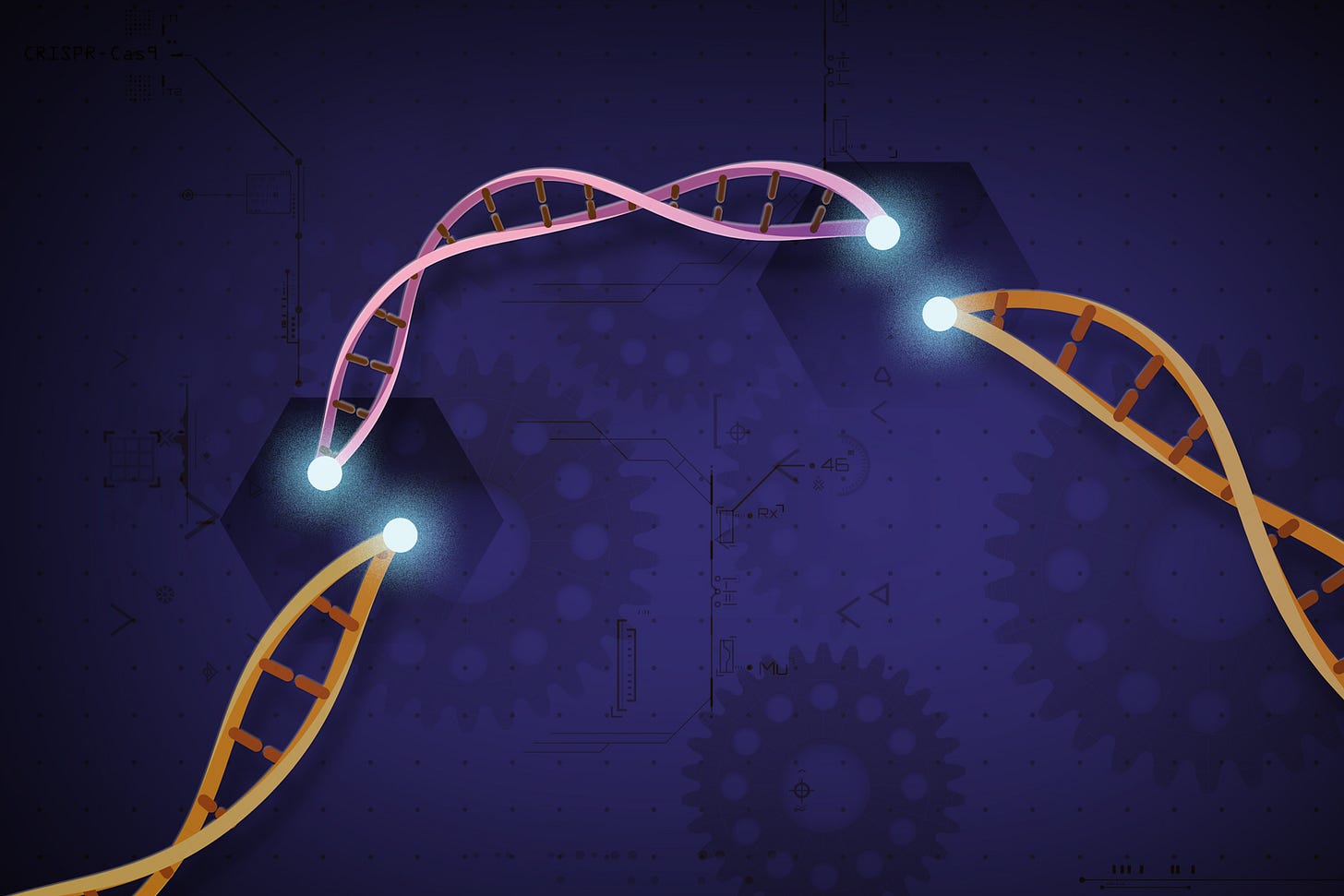
Origins of CRISPR
Discovery and Initial Observations
The story of CRISPR began in the late 1980s when researchers observed unusual repeating sequences in the DNA of Escherichia coli and other bacteria. These sequences, characterized by their regular intervals and palindromic nature, were initially considered a curiosity with no known function. Yoshizumi Ishino and colleagues were among the first to report these sequences in 1987, noting their presence but could not determine the function.
The scientific community remained puzzled by these repetitive sequences for over a decade. It wasn't until 2005 that a groundbreaking discovery shed light on their function. Francisco Mojica and his team observed that these CRISPR sequences were interspaced with unique sequences that matched fragments of viral genomes. They proposed that these sequences were part of an adaptive immune system in bacteria and archaea, allowing them to "remember" and defend against viral infections.
Evolutionary Significance
CRISPR-Cas systems are found in approximately 40% of sequenced bacterial genomes and 90% of archaeal genomes, reflecting their functional and evolutionary significance. These systems provide bacteria and archaea with acquired immunity, enabling them to capture and incorporate segments of viral DNA into their genomes. This process allows these microorganisms to recognize and defend against future infections by the same viruses.
The evolutionary advantage conferred by CRISPR-Cas systems is profound. In environments where viral infections are rampant, adapting and remembering past infections provides a critical survival advantage. This evolutionary arms race between bacteria and their viral predators has driven the diversification and optimization of CRISPR-Cas systems, resulting in various CRISPR types and subtypes with specialized functions and mechanisms.
Mechanism of CRISPR-Cas Systems
Structure and Function
CRISPR loci consist of short, repetitive DNA sequences interspaced with unique "spacer" sequences derived from past viral infections.
These loci are transcribed into RNA molecules that guide Cas (CRISPR-associated) proteins to target and cut viral DNA. The CRISPR-Cas immune response can be divided into three main stages:
Adaptation: When a new virus infects a bacterium, a segment of the viral DNA is captured and inserted into the CRISPR array as a new spacer. Cas proteins facilitate this process, which recognizes and cleaves the viral DNA, incorporating a small fragment into the CRISPR locus.
Expression: The CRISPR array is transcribed into a long RNA molecule and processed into shorter CRISPR RNAs (crRNAs) containing the viral sequences. These crRNAs are then assembled with Cas proteins to form an RNA-protein complex.
Interference: The crRNAs guide Cas proteins to the matching viral DNA, where the Cas proteins cleave the DNA, neutralizing the threat. This sequence-specific targeting and cleavage of viral DNA is the hallmark of the CRISPR-Cas immune system.
Evolutionary Adaptations
Different CRISPR-Cas systems have evolved, each with unique mechanisms and proteins involved in the immune response. The two major classes of CRISPR-Cas systems, Class 1 and Class 2, differ in their structural complexity and the number of proteins involved. Class 1 systems utilize multiple Cas proteins to form a complex that targets and cleaves DNA, whereas Class 2 systems rely on a single, multifunctional protein like Cas9 or Cpf1.
These variations reflect the evolutionary arms race between bacteria and their viral predators. For instance, some viruses have evolved mechanisms to evade CRISPR-Cas systems, such as anti-CRISPR proteins that inhibit Cas activity. In response, bacteria have evolved countermeasures, leading to a dynamic and ongoing co-evolutionary process.
CRISPR and Evolutionary Genomics
Studying Evolutionary Processes
CRISPR technology has revolutionized our ability to study evolutionary biology. Researchers can investigate the function of specific genes and their role in evolutionary processes by enabling precise manipulation of genes. For instance, CRISPR has introduced mutations into model organisms like fruit flies (Drosophila melanogaster) and zebrafish (Danio rerio), providing insights into developmental biology, evolutionary genetics, and the principles of gene function.
In one notable study, researchers used CRISPR to knock out genes in fruit flies to study their role in development and behavior. This approach has helped identify genes involved in sensory perception, mating behavior, and other traits. Similarly, CRISPR has been used to study the genetic basis of adaptation in other organisms, such as identifying genes that confer resistance to environmental stressors.
Experimental Evolution
CRISPR also facilitates experimental evolution studies, where researchers can create populations with specific genetic modifications and observe how these changes affect survival and reproduction over generations. This approach helps to identify the genetic basis of adaptation and evolutionary fitness. For example, researchers have used CRISPR to introduce specific mutations into bacterial populations and then tracked the evolutionary trajectories of these populations under different environmental conditions.
This method allows scientists to experimentally test evolutionary hypotheses and better understand the mechanisms driving evolution. By observing how genetic changes affect an organism's fitness in various environments, researchers can infer the selective pressures and adaptive strategies that shape evolutionary outcomes.
Ancient DNA and Evolutionary History
Another exciting application of CRISPR is in the study of ancient DNA. By editing the genomes of living organisms to resemble those of extinct species, scientists can gain insights into these long-lost organisms. This method, known as "de-extinction," involves using CRISPR to insert ancient DNA sequences into the genomes of closely related living species.
For instance, researchers have proposed using CRISPR to insert woolly mammoth genes into the genome of modern elephants, potentially reviving traits such as cold resistance and thick fur. While the ethical and practical challenges of de-extinction are significant, this approach offers a unique opportunity to study the evolutionary adaptations of extinct species and their potential responses to current environmental changes.
Impact on Biodiversity and Conservation
Genetic Rescue of Endangered Species
CRISPR offers new possibilities for biodiversity conservation. Genetic rescue, where beneficial genes are introduced into endangered populations, can enhance genetic diversity and improve resilience against diseases and environmental changes. For example, CRISPR has been proposed to help save the endangered black-footed ferret by introducing genetic diversity lost due to inbreeding.
In one notable effort, scientists are using CRISPR to edit the genes of the black-footed ferret to increase genetic diversity and resistance to diseases like sylvatic plague. By carefully selecting and introducing beneficial genetic variants, researchers aim to enhance these endangered animals' survival and reproductive success, thereby bolstering the population size.
Ethical Considerations
One of the most controversial applications of CRISPR is de-extinction, the idea of bringing back extinct species. While technically feasible, this concept raises numerous ethical and ecological questions. Reviving extinct species like the woolly mammoth could provide insights into ancient ecosystems. Still, it also poses risks to current biodiversity and raises concerns about the welfare of de-extinct organisms.
Ethical considerations include the potential impact on existing ecosystems, the welfare of de-extinct animals, and the allocation of resources to de-extinction efforts versus the conservation of endangered species. Moreover, introducing de-extinct species into modern ecosystems could have unforeseen consequences, potentially disrupting current species interactions and ecological balances.
Future Prospects and Challenges
Advancements in CRISPR Technology
The future of CRISPR is bright, with ongoing advancements aimed at improving its precision, efficiency, and range of applications. Newer versions of CRISPR, such as CRISPR-Cas13, which targets RNA instead of DNA, and base editors that can make single-nucleotide changes without cutting the DNA, are expanding the toolkit available to researchers.
CRISPR-Cas13, for example, allows researchers to target and degrade specific RNA molecules, offering potential applications in antiviral therapies and gene regulation studies. On the other hand, base editors enable precise editing of individual DNA bases, allowing for the correction of point mutations without introducing double-strand breaks. These advancements are pushing the boundaries of what is possible with gene editing and opening new avenues for research and therapeutic applications.
Ethical and Regulatory Challenges
As CRISPR technology advances, ethical and regulatory challenges become increasingly important. Issues such as off-target effects, unintended consequences, and the potential for germline editing in humans require careful consideration and robust regulatory frameworks. Ensuring responsible use of CRISPR technology will be crucial to maximizing its benefits while minimizing risks.
Off-target effects, where CRISPR unintentionally edits genome regions similar to the target site, can lead to unintended mutations and potential health risks. Advances in CRISPR technology aim to reduce these off-target effects, but regulatory oversight is essential to ensure the safety and efficacy of CRISPR-based therapies. Additionally, ethical considerations surrounding germline editing, which involves making heritable changes to the human genome, necessitate rigorous ethical debates and international consensus. Moreover, human gene editing could closely resemble, or even be considered, eugenics, a direction we should avoid taking.
CRISPR in Medicine and Agriculture
Beyond evolutionary genomics, CRISPR's potential applications in medicine and agriculture are vast. CRISPR is being used to develop gene therapies for genetic disorders, cancer, and infectious diseases in medicine. For example, clinical trials are underway to use CRISPR to correct genetic mutations responsible for diseases like sickle cell anemia and cystic fibrosis.
CRISPR can make crops more resistant to pests, diseases, and environmental stresses in agriculture, potentially improving food security and sustainability. Researchers use CRISPR to develop crops with enhanced nutritional profiles, increased yield, and resistance to climate change-related stressors. These advancements can address global challenges such as food scarcity and malnutrition.
Conclusion
CRISPR, from a bacterial immune system to a revolutionary gene-editing tool, is a testament to the power of scientific discovery and innovation. As we continue to explore its potential, CRISPR holds promise for unlocking new insights into evolutionary biology, improving biodiversity conservation, and transforming medicine and agriculture. However, the ethical and regulatory challenges must be addressed to ensure this powerful technology is used responsibly and for the greater good.
References
Ishino, Y., et al. (1987). "Nucleotide sequence of the iap gene, responsible for alkaline phosphatase isozyme conversion in Escherichia coli, and identification of the gene product." Journal of Bacteriology, 169(12), 5429-5433.
Mojica, F. J., et al. (2005). "Intervening sequences of regularly spaced prokaryotic repeats derive from foreign genetic elements." Journal of Molecular Evolution, 60(2), 174-182.
Barrangou, R., et al. (2007). "CRISPR provides acquired resistance against viruses in prokaryotes." Science, 315(5819), 1709-1712.
Grissa, I., Vergnaud, G., & Pourcel, C. (2007). "The CRISPRdb database and tools to display CRISPRs and to generate dictionaries of spacers and repeats." BMC Bioinformatics, 8(1), 172.
Doudna, J. A., & Charpentier, E. (2014). "The new frontier of genome engineering with CRISPR-Cas9." Science, 346(6213), 1258096.
Makarova, K. S., et al. (2011). "Evolution and classification of the CRISPR-Cas systems." Nature Reviews Microbiology, 9(6), 467-477.
Housden, B. E., et al. (2015). "Loss-of-function genetic tools for animal models: cross-species and cross-platform differences." Nature Reviews Genetics, 16(1), 38-44.
Blount, Z. D., Barrick, J. E., & Lenski, R. E. (2012). "Genomic analysis of a key innovation in an experimental Escherichia coli population." Nature, 489(7417), 513-518.
Pääbo, S. (2014). "The human condition-a molecular approach." Cell, 157(1), 216-226.
Novak, B. J. (2018). "De-extinction." Genes, 9(11), 548.
Shapiro, B. (2016). "Pathways to de-extinction: how close can we get to resurrection of an extinct species?" Functional Ecology, 31(5), 996-1002.
Church, G. M., & Regis, E. (2014). Regenesis: How Synthetic Biology Will Reinvent Nature and Ourselves. Basic Books.
Abudayyeh, O. O., et al. (2017). "RNA targeting with CRISPR-Cas13." Nature, 550(7675), 280-284.
Gaudelli, N. M., et al. (2017). "Programmable base editing of A•T to G•C in genomic DNA without DNA cleavage." Nature, 551(7681), 464-471.
Jinek, M., et al. (2012). "A programmable dual-RNA–guided DNA endonuclease in adaptive bacterial immunity." Science, 337(6096), 816-821.
Komor, A. C., et al. (2016). "Programmable editing of a target base in genomic DNA without double-stranded DNA cleavage." Nature, 533(7603), 420-424.
Brokowski, C., & Adli, M. (2019). "CRISPR ethics: Moral considerations for applications of a powerful tool." Journal of Molecular Biology, 431(1), 88-101.